Introduction
Materials and Methods
1. Plant materials and germination condition
2. Light quality
3. Germination test
4. Statistical analysis
Results and Discussion
1. Percent of germination
2. Mean germination time
3. Germination rate
4. Mean daily germination
5. Germination energy
Introduction
The carrot (Daucus carota L.) is a biennial root vegetable known for its vibrant color, distinctive flavor, and numerous health benefits (Que et al., 2019). According to KOSIS (2023), carrots in Korea were cultivated on 2,486 hectares, yielding a productivity of 88,063 tons in 2023, accounting for 10.4% of total root vegetable area and 6.7% of total root vegetable production. As one of the most widely cultivated vegetables, carrots provide consumers with a rich supply of nutrients at an affordable price. They are recognized among the top nine vegetables for their high nutritional value and cost-effectiveness (Drewnowski, 2010). Carrots are an excellent source of beta-carotene, which serves as a precursor to vitamin A. Additionally, they contain a significant amount of vitamin B compared to other commonly consumed vegetables, thereby providing a valuable source of dietary fiber as well (Arscott and Tanumihardjo, 2010; Drewnowski, 2010; Suchánková et al., 2015). Consequently, the demand for and consumption of carrots are on the rise, driven by increasing awareness of their health benefits and nutritional value (Selvakumar et al., 2019).
Plant development typically commences with seed germination and progresses through distinct stages, including the seedling stage, vegetative growth, and ultimately reproductive growth (Graeber et al., 2012). Germination marks the first stage of the plant life cycle and influences the overall growth of the plant; higher germination rates increase the overall growth potential of crops (Finch-Savage and Bassel, 2016). Robust seed germination is crucial for high-quality crop production. The uniformity and speed of seed germination exert a substantial influence on the final crop yield, quality, and ultimately the income of farmers (Mirmazloum et al., 2020). Accelerating crop growth by promoting rapid germination in energy-intensive plant factories is economically advantageous for farmers.
Light plays a crucial role in plant growth by influencing their development, regulating metabolic processes, and promoting the synthesis of hormones and enzymes (Vieira et al., 2010). The typical cultivation process of plants involves sequential stages, such as sowing, cultivation, and harvesting. Extensive research is actively being undertaken on the light environment required for cultivation following planting. However, research on the light environmental conditions from the sowing to germination stages is currently limited. Moreover, there is a lack of investigation into whether the light environment conditions during the germination and cultivation stages are consistent for a given crop.
Light sensitivity varies between plant species, leading to negative, neutral, or positive photomorphogenic responses (Sousa et al., 2008). Researchers investigating Stevia rebaudiana Bertoni seeds sought to identify appropriate light qualities to improve the species’ low germination rates (Simlat et al., 2016). According to the findings of this study, Stevia rebaudiana Bertoni seeds exhibited a significant increase in germination rates under blue LED light, whereas red LED light had a negative effect on germination, similar to conditions in darkness. Lettuce is a typical photoblastic species, demonstrating distinct germination responses to varying light qualities, as evidenced in the research conducted by Hwang et al. (2008). In white and red light, germination energy was notably high, whereas the germination rate was significantly lower under far-red light. Similarly, the impact of light quality varies across different crops. Carrots are generally recognized as photoblastic seeds; however, research regarding the optimal light spectrum for germination and the adequacy of existing light conditions for cultivation post-planting remains insufficient.
This study aimed to explore the optimal light conditions for carrot germination and post-germination growth stages. In addition, the study investigated whether the light environment during germination was consistent with that during post-germination growth. If the light requirements for both the germination and cultivation stages are identical, there is no need for additional space to accommodate separate light environments. However, if the required light conditions differ, separate settings are required to optimize the environment for each stage, thereby enhancing the efficiency of space and resource utilization. This study explored whether the light conditions required for carrot germination are consistent with those required during cultivation following planting. It also identifies the optimal light conditions from this perspective.
Materials and Methods
1. Plant materials and germination condition
Seeds of the carrot (Daucus carota L.) variety (baby carrot, Sakata Seed Corporation, Japan) were used in this study. The experiment was conducted in a controlled-environment plant factory situated at Jeju National University. The laboratory temperature was maintained at 22 ± 1.9°C, whereas the Relative humidity was set to 60 ± 6%. For each treatment, 100 seeds were arranged on top of filter paper (Whatman No. 2, GE Healthcare, Buckinghamshire, UK) placed in a Petri dish. Germination tests were conducted using a completely randomized design, with 100 seeds per treatment and four replications. To maintain consistent experimental conditions, plastic containers (22.5 × 31.5 × 28.0 cm) were equipped with DC fans (5 × 5 cm). This was done to ensure smooth airflow and humidity control by maintaining a certain level of distilled water at the bottom of the container (Fig. 1). The environmental control system was specifically designed to maintain optimal conditions for plant growth, with the temperature and humidity continuously monitored and adjusted using dedicated sensors. Germination rate and speed were recorded daily, and the germination characteristics of each treatments were compared and analyzed.
2. Light quality
In this study, the photosynthetic photon flux density (PPFD) of LED lighting (HT204, ESLEDs, Korea) was measured using spectral PAR meter (PG200N, UPRtek, Taiwan). The LED lighting system (600 × 28 × 16 cm) was specifically designed to maintain a uniform and uninterrupted light cycle within the experimental environment. Specifically, a photoperiod of 12 h daytime and 12 h nighttime was established to mimic the natural growth cycle of the plants. The light intensity was fixed at 200 μmol·m-2·s-1, and a quantum sensor (LI-190R, LI-COR, Lincoln, NE, USA) was used to measure PPFD. LED lighting emitted a mixed spectrum, consisting of red light (600–700 nm), green light (500–600 nm), and blue light (400–500 nm), to evaluate the effects of light at various wavelengths on plant growth. Experiments were conducted using various light ratios. These ratios comprised five conditions: R:G:B = 10:0:0, 8:0:2, 7:0:3, 6:1:3, and 0:0:10. Complete darkness was used as the control group for comparison (Fig. 2). This allowed for the analysis of the influence of each wavelength ratio on the germination of carrot (D. carota L.) seeds. To ensure the accuracy of the experimental environment, partitions were installed within the plant factory to separate each treatment. These partitions were implemented to isolate the light environment of each treatment, ensuring that they did not interfere with one another and enabling independent experimentation. Carrot seed germination rates in each treatment were observed in a carefully controlled experimental environment. The data were then analyzed to evaluate the effects of various light spectra on germination.
3. Germination test
The experiment was conducted from March 25, 2024, to April 11, 2024, for a total of 16 d. For the first 3 d of the experiment, observations were made at 24-h intervals, with particular emphasis on the early stages of germination. Subsequently, for the following 6 d, observations were conducted at 12-h intervals to analyze germination speed more precisely. During the final 7 d, observations were once again made at 24-h intervals. This change in the observation interval was aimed at effectively monitoring significant changes occurring at each stage of the germination process. Seeds with cotyledons exceeding a length of 2 mm were extracted from the Petri dishes, and the number was recorded.
Germination characteristics were investigated using indicators such as percentage of germination (PG), germination energy (GE), mean germination time (MGT), germination rate (GR), and mean daily germination (MDG). These indicators were calculated using the formulas presented in Table 1.
Table 1.
The method of calculating seed germination.
Studied indices | Calculation formula | Unit of measurement | Reference |
Percent of germination | % | ||
Germination energy | - | ||
Mean germination time | day | Chae et al., 2006 | |
Germination rate | - | ||
Mean daily germination | seeds/day |
4. Statistical analysis
The experiment was conducted using a completely randomized design with four replicates. The statistical analysis was performed using Duncan’s multiple range test at a 95% confidence level using SAS (SAS 9.1, SAS Institute Inc., Cary, NC, USA). The graphs were generated using SigmaPlot software (10.0, Systat Software, Inc., Chicago, IL, USA).
Results and Discussion
1. Percent of germination
PG is an indicator that calculates the seedling emergence of seeds as a percentage, representing the proportion of seeds that have successfully germinated out of the total number of seeds (Chae et al., 2006). It is used to evaluate the germination performance of plants and provides crucial insights into seed quality, storage conditions, treatment methods, and environmental conditions. A high PG indicates that the germination environment is suitable, which is of great importance in agriculture, horticulture, and ecological restoration projects (Bewley and Black, 1994). The PG can be influenced by various environmental and biological factors.
The R:G:B = 0:0:10 treatment exhibited a rapid increase in the PG, exceeding 80% germination by Day 5 and maintaining over 90% germination after Day 10 (Fig. 3). This indicates that green light may be effective in promoting germination, whereas blue light has a more positive effect on germination compared to red light. The dark treatment showed over 70% germination by Day 6, ultimately maintaining a PG of 87%. This demonstrates that germination is possible even in the absence of light and that high GRs can be achieved under certain conditions. In particular, the PG under dark conditions, although lower than that under blue light alone, was much higher than that under red light alone. This suggests that certain inherent factors that promote germination remain active even in the absence of light. Carrots are classified as light germinators (Lee, 2013), and as demonstrated in this study, germination occurs even under dark conditions. The R:G:B = 6:1:3 treatment exhibited the lowest PG during the initial stages of germination, reaching 41% by Day 5, and ultimately recording approximately 60% germination. This indicates that conditions with a high proportion of green light inhibit germination, resulting in a decreasing trend in the PG as the proportion of green light increases. The final PG values decreased sequentially in the R:G:B = 7:0:3, 8:0:2, and 10:0:0 treatments (86.25, 83, and 75%, respectively), thereby indicating that the germination-promoting effect of blue light was inhibited as the proportion of red light increased.
This study demonstrated the substantial influence of light quality on plant germination, specifically emphasizing the stimulating effect of blue light and the contrasting inhibitory effects of red and green light. Research showing the positive influence of blue light on crop germination has also been reported in Stevia and Nicotiana tabacum L. (Cocco et al., 2022; Simlat et al., 2016). However, the precise role of blue light in carrot seed germination and the underlying mechanisms are not yet well understood.
In general, light encompasses the act of detecting light signals through specialized photoreceptors known as phytochromes (Neff, 2012). Phytochromes detect light to regulate seed germination, which includes processes such as seed coat and endosperm rupture and root protrusion (Kim et al., 2014). Cryptochrome, a type of photoreceptor, is activated by blue light and hypothesized to promote germination (Ahmad and Cashmore, 1993; Lin, 2000). Upon activation by blue light, cryptochrome modulates the expression of various genes and proteins that initiate germination (Guo et al., 1999). The activated cryptochrome in the blue light treatment acts through several mechanisms to promote seed germination. First, cryptochrome inhibits the activity of phytochrome-interacting factors (PIFs) (Shen et al., 2005). PIFs activate genes that promote germination under dark conditions. However, cryptochrome, activated by blue light, promotes PIF degradation, thereby inhibiting the expression of these inhibitory genes (Leivar and Quail, 2011). Second, blue light promotes gibberellin (GA) synthesis and suppresses abscisic acid (ABA) signaling to promote germination (Seo et al., 2009). GA promotes the degradation of DELLA proteins, thereby inhibiting the expression of ABI5, an inhibitory factor that promotes germination by weakening the ABA signal (Zentella et al., 2007). During this process, cryptochrome, activated by blue light, plays an important role in regulating the balance between GA and ABA (Finkelstein et al., 2008). In conclusion, blue light promotes seed germination through the cryptochrome photoreceptor. This process involves several physiological mechanisms, including inhibiting PIF transcription factors (Shen et al., 2005), promoting GA synthesis (Seo et al., 2009), and suppressing ABA signaling (Finkelstein et al., 2008).
The observation that the germination rate under dark conditions is lower than that when treated with only blue light, but significantly higher than that when treated with only red light, indicates the presence of inherent germination-promoting factors, even under dark conditions. This suggests that carrot seeds have the inherent ability to undergo natural germination without being dependent on light. This signifies an ecological strategy in which seeds can survive and propagate even in dark environments where light is limited. Although carrots are typically considered to be photoblastic (Lee, 2013), this study presents contrasting results. Because germination occurred regardless of the presence of light, the carrot seeds used in this experiment could be considered light-independent. This suggests a shift in the characteristics of seeds during the carrot breeding process, leading to the development of light-independent seeds; however, further research on this topic is required.
2. Mean germination time
The MGT is the average number of days required for all germinated seeds to sprout (Chae et al., 2006). This metric measures the average time from initiation to completion of germination and is used to evaluate the germination speed. Generally, a low MGT value indicates rapid germination, whereas a high value indicates delayed germination. The time taken to reach a specific PG in each treatment was determined by evaluating the effect of light conditions on germination speed (Fig. 4). (A) represents the time required to reach a PG of 30%, (B) represents the time required to reach a 50% PG, and (C) represents the time required to reach an 80% PG. Analysis of the time taken for each treatment to reach a 30% PG revealed that the R:G:B = 0:0:10 treatment required the shortest time of approximately 2.9 d, indicating vigorous initial germination.
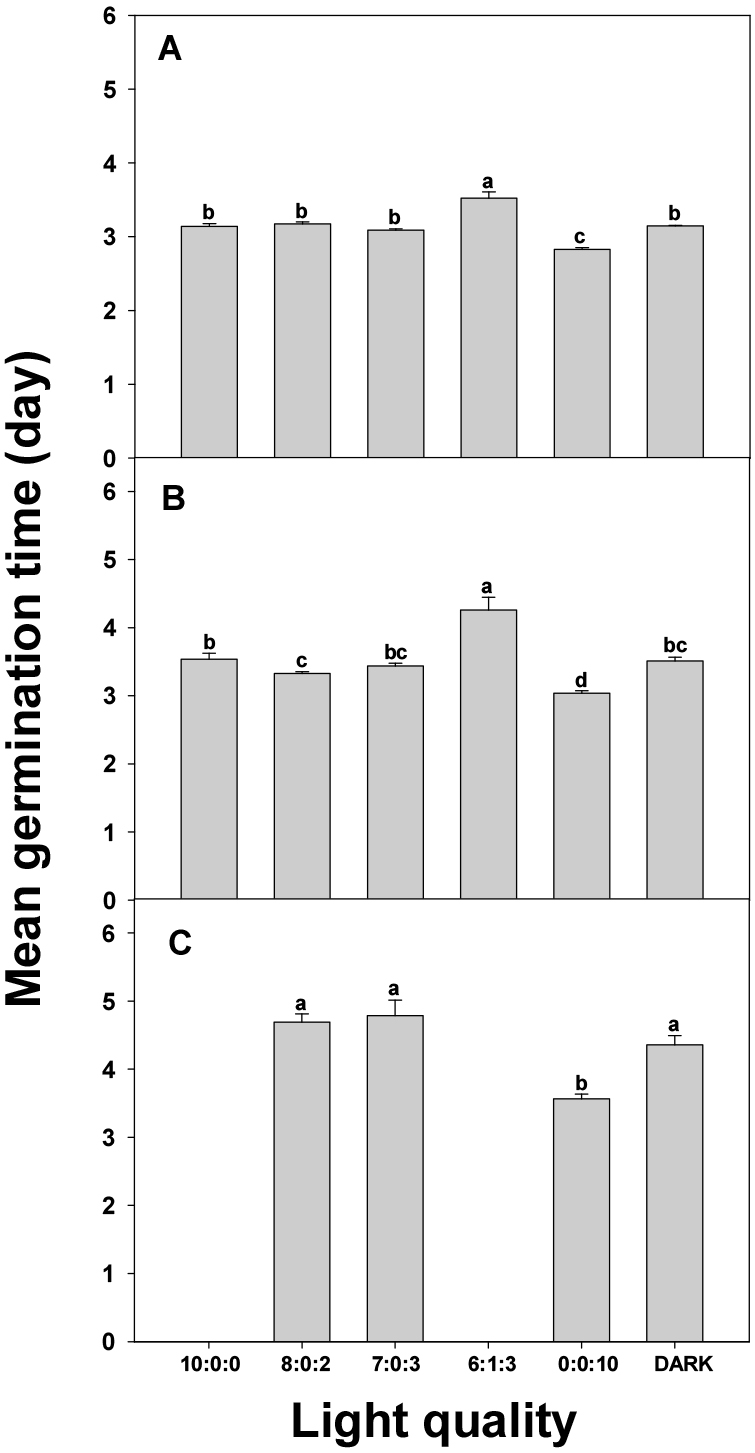
Fig. 4
Mean germination time of carrot seeds under different light quality. A, B, C represent the time taken to reach germination rates of 30%, 50%, and 80%, respectively. Vertical bars indicate the standard error of the means (n = 4). Values for the same parameter with different letters indicate significant differences at the 5% level.
In contrast, the R:G:B = 6:1:3 treatment recorded the longest time of approximately 3.3 d, indicating slow initial germination. Other treatments, R:G:B = 10:0:0, 8:0:2, 7:0:3, and dark, recorded times of 3.1 d, 2.8 d, 3.0 d, and 3.1 d, respectively, indicating moderate durations. Even when the PG reached 50%, the R:G:B = 0:0:10 treatment recorded the shortest germination time of approximately 3.0 d, demonstrating its effectiveness in promoting germination. Conversely, the R:G:B = 6:1:3 treatment required the longest treatment time of approximately 4.0 d. R:G:B = 10:0:0, 8:0:2, 7:0:3, and the DARK treatment recorded times of 3.2 d, 3.4 d, 3.6 d, and 3.5 d, respectively, indicating moderate durations. At an 80% PG, the R:G:B = 0:0:10 treatment recorded the shortest time at approximately 3.6 d, indicating its effectiveness in both the initial and overall germination processes. The R:G:B = 7:0:3 treatment had the longest treatment time of approximately 4.2 d. The R:G:B = 8:0:2 and DARK treatments were recorded for 3.9 d each, indicating moderate durations. The R:G:B = 10:0:0 and R:G:B = 6:1:3 treatments did not yield values as they did not reach 80% PG s, with final PG s of 60.75% and 75%, respectively.
3. Germination rate
GR represents the sum of the daily germination speeds for all seeds, indicating the overall speed and efficiency of the germination process (Chae et al., 2006), which is a crucial factor in agricultural production. Rapid germination reduces susceptibility to pests and diseases and provides an advantage in early-growth competition (Bradford, 2002).
The condition with the highest GR was R:G:B = 0:0:10 (Fig. 5). In this study, the GR rapidly increased, reaching its peak at approximately 10 d, and then maintained a stable rate. These results indicate that blue light has a highly positive effect on seed germination. The second-highest GR was observed in the dark. Although the initial GR was slower than that under blue light conditions, it sharply increased after approximately 5 d and maintained a stable rate after 15 d. This demonstrates that seeds can germinate effectively even in environments that are completely devoid of light. The R:G:B ratios of 8:0:2, 7:0:3, and 10:0:0 did not show any significant differences. Although the initial GR was relatively lower than that of R:G:B (0:0:10), it increased over time and stabilized. This suggested that the measured light conditions had some influence on the GR. The lowest GR was observed for an R:G:B ratio of 6:1:3. Although the GR was somewhat slower until Day 5, it steadily increased thereafter, maintaining a stable germination rate after 10 d. This indicates that a mixture of different light wavelengths affects the germination rate.
In summary, by comparing the germination rates of seeds under various light conditions, it can be concluded that blue light was the most effective. These findings enable the identification of optimal germination conditions by adjusting environmental variables such as light quality. This could directly enhance agricultural productivity (Finch-Savage and Leubner-Metzger, 2006).
4. Mean daily germination
The MDG indicates the average number of seeds germinated per day and plays a crucial role in evaluating the speed and uniformity of seed germination. This metric allows for the assessment of seed vigor, which is significant in agriculture and horticulture because seed quality directly influences yield and uniformity (Djavanshir and Pourbeik, 1976). All the treatments showed a sharp increase on Day 3 (Fig. 6). The MDG was prominently observed between Days 3 and 7 under various light treatment conditions. The peak MDG value observed in each treatment occurred earliest at around Day 3.9 with a value of 16.3 in R:G:B = 10:0:0, which was the highest among all treatments, whereas in all other treatments, it occurred around Day 4.4 with lower values. The early occurrence and high value of the peak MDG indicate rapid and concentrated germination under these conditions, suggesting that specific light conditions effectively promote germination. When the peak MDG occurs early and reaches a high value, light conditions induce germination rapidly and efficiently (Bewley and Black, 1994). Rapid and uniform germination confers advantages in resource competition during the initial growth stages, potentially exerting positive effects throughout the growth of the plant. Therefore, the treatment with R:G:B = 0:0:10 had the highest MDG, indicating that blue light most effectively promoted germination.
In contrast, the R:G:B = 10:0:0 and blue light mixture conditions (R:G:B = 8:0:2, 7:0:3) exhibited high initial GRs but showed a tendency for a slight decrease in GRs over time. The green light mixture (R:G:B = 6:1:3) had the lowest initial GR, with a relatively slow increase in the GR. The dark treatment showed a slightly lower initial GR; however, a relatively high germination rate was observed after day four. In particular, the higher germination rate under dark conditions compared to that when treated with blue light only, but significantly higher than that when treated with red light only, indicates the presence of inherent germination-promoting factors, even under dark conditions. These findings underscore the significant impact of light quality on plant germination, emphasizing the contrasting effects of blue light on germination, whereas red and green light inhibit germination. These results provide valuable insights into the optimization of germination rates through light manipulation in agriculture and horticulture.
5. Germination energy
GE is an indicator of the speed and uniformity of seed germination; a higher germination energy signifies that a greater number of seeds germinate uniformly within a shorter period (Zhang et al., 2020). It is closely related to the initial vigor of seeds and is crucial for predicting the overall growth potential of crops (Domin et al., 2020). By evaluating GE, we can understand the germination characteristics of seeds under specific environmental conditions, thereby enabling us to create optimal germination environments to enhance crop productivity.
A comparison of carrot GE under various light conditions is shown in Fig. 7. First, the results on Day 3 of the experiment (A) demonstrated that GE was the highest in the R:G:B = 0:0:10 treatment. Conversely, relatively lower GE was observed under R:G:B = 10:0:0, 8:0:2, 7:0:3, and 6:1:3 conditions, particularly under the 0:0:10 condition, which significantly outperformed all other conditions. This indicated the highly effective promotion of carrot germination by blue light during the initial germination stage. Similarly, on Day 6 of the experiment (B), R:G:B = 0:0:10 continued to exhibit the highest GE. The R:G:B = 10:0:0, 8:0:2, and 7:0:3 conditions showed similar levels of GE, whereas the dark conditions yielded comparable results. Again, the R:G:B = 0:0:10 condition significantly outperformed the other conditions, highlighting the sustained positive effect of blue light on germination throughout the process. The results on Day 9 of the experiment (C) indicate an overall increase in GE. The R:G:B = 0:0:10 condition maintained the highest GE, significantly outperforming other conditions. The R:G:B = 7:0:3 condition exhibited significantly lower GE. This underscores the continuous positive influence of blue light on carrot germination, demonstrating its superior effectiveness compared to other light conditions.
In conclusion, the evaluation of carrot GE under various light conditions consistently showed that R:G:B = 0:0:10 yielded the highest GE at all time points. Conversely, conditions that included red light resulted in relatively lower GE. Although the dark condition initially showed lower GE levels, they showed an increasing trend over time. These results strongly indicate the positive impact of blue light on carrot germination, particularly exerting a significantly positive influence on the initial GE compared to other treatments. This suggests that the utilization of blue light may be advantageous for efficient germination when selecting light conditions.
Another reason why germination indicators were superior under dark conditions following blue light exposure may be that the carrot seeds used in this experiment were coated with a blue substance. Because the seeds were blue, it is possible that they reflected blue light and did not absorb it sufficiently. The presence of pigments, such as anthocyanins and tannins, in seed coatings can greatly alter light-reaching photoreceptors (Goggin and Seadman, 2012; Hendricks et al., 1968). In Lolium rigidum seeds coated with a yellow-brown coating, approximately 80% of the white light was blocked, and blue and green light were filtered more effectively than red and far-red light (Goggin and Seadman, 2012). Therefore, it is possible that in this treatment, the seeds did not absorb blue light sufficiently and were perceived to be in an environment similar to darkness.
The R:G:B = 6:1:3 treatment was the least suitable for carrot germination among all indicators, suggesting that green light inhibited the role of blue light in promoting germination. Green light may interfere with the effects of blue light and reduce the GR. Because research on the effects of green light on plant germination is relatively scarce, it is not yet fully understood. However, interesting recent findings have also been presented. When green light was investigated together with blue light, it was found that green light could deactivate photoreceptors activated by blue light, leading to negative effects on germination, as confirmed in Arabidopsis thaliana (Bouly et al., 2007). Blue light activates cryptochromes (Ahmad et al., 1996), the photoreceptors in plants that primarily respond to blue light and trigger essential physiological changes during seed germination. The flavin pigment of cryptochromes exists in an oxidized form (FAD). When stimulated by blue light, FAD is reduced to FADH•, which activates the signal state of the receptor (green absorption state). Illumination with green light generates a fully reduced form of the pigment (FADH–), which deactivates the receptor (Bouly et al., 2007; Folta and Maruhnich, 2007). This indicated that green light can reverse the activity induced by blue light, thereby regulating the physiological responses of plants. Therefore, the lowest germination indicators in this treatment suggest a reversal of the blue light effect by green light. According to Sung et al. (2024), the R:G:B = 6:1:3 condition for carrot growth following planting with LEDs was demonstrated to be the most suitable. However, in the present study, carrot germination showed the lowest indicators at an R:G:B ratio of 6:1:3. Therefore, it can be concluded that different lighting conditions should be set for the germination and post-planting stages of carrot cultivation. Although considerable research has been conducted on setting the lighting environment for post-planting growth, in-depth research on the lighting conditions during the germination stage is required. In addition, it is important to meticulously examine the transition point from the germination stage to post-planting growth from a plant’s physiological perspective to accurately determine the optimal timing for optimizing environmental changes.
In future research, a more precise understanding of the germination mechanism of carrot seeds under various light conditions and seed coating methods is needed. This will enable the development of strategies to optimize carrot germination and explore methods to increase germination success rates under various environmental conditions. In particular, research is needed to clarify the differences in lighting environments between the germination and post-planting growth stages and to optimize the transition point.