Introduction
Materials and Methods
1. Plant Materials, Growth Conditions, and Treatments
2. Shoot Fresh Weight and Leaf Water Potential
3. Sugar Content
4. Total Phenolic Content, Total Flavonoid Content, and Antioxidant Capacity
5. Protective effect of cytotoxicity in mugwort
6. Data Analysis
Results and Discussion
Comparison with Osmotic Stress
1. Shoot Fresh Weight and Leaf Water Potential
2. Total Phenolic Content, Total Flavonoid Content, and Antioxidant Capacity
3. Protective Effect of Cytotoxicity
Comparison with Biotic Stress
1. Shoot Fresh Weight and Sugar Analysis
2. Total Phenolic Content and Antioxidant Capacity
Conclusion
Introduction
Sucrose (suc) is a disaccharide composed of glucose (glu) and fructose (fru). Since both anomeric groups are involved in the glycosidic linkage, suc is a non-reducing sugar with limited chemical reactions. Therefore, it is a stably transported carbohydrate in plants, and is used as the primary form for carbohydrate translocation (Mun and Yu, 2015). Suc plays important roles in plant growth and development because it is used as a raw material to synthesize essential compounds, such as protein, cellulose, and starch in plants, and it also regulates the expression of microRNAs and transcription factors by suc signaling (Rook et al., 1998). It can directly affect plants' primary and secondary metabolism (Tognetti et al., 2013). Suc signaling that affects primary metabolism is the inhibition of the expression of plastocyanin (mobile electron carrier in photosynthesis), such that it reduces photosynthesis of Arabidopsis and promotes the patatin-1 promoter (expressing the patatin gene) activity of potato tubers (Jefferson et al., 1990; Dijkwel et al., 1996). On the other hand, suc signaling in secondary metabolism induces MYB75/PAP1, which is necessity for the expression of the suc-mediated dihydroflavonol reductase gene and anthocyanin biosynthesis genes of Arabidopsis and increases the activity of phenylalanine ammonia-lyase (PAL) to enhance the total phenol content in broccoli sprouts. Various studies have reported that suc signaling can induce positive effects that promote bioactive compounds (Teng et al., 2005; Solfanelli et al., 2006; Julia et al., 2010; Guo et al., 2011).
Our previous study demonstrated that the plant absorbed suc in a nutrient solution via the roots of two different plant species (mugwort and kale), and effectively increased the bioactive compounds of both plants (Yeom et al., 2019). However, there is several points to consider before supplying the suc through the roots of these plants to ensure that the bioactive compounds have been promoted through signaling. First, suc treatment in the deep-flow technique (DFT) system could increase the concentration of solutes, such that the roots of the plant can be exposed to decreased water potential (Amrhein et al., 2013). Second, there are various microorganism in hydroponic systems, and suc treatments can promote the growth of harmful microorganism, such as bacteria and fungi; consequently, it can induce biotic stress (Gilberto, 2011). Akula and Ravishankar (2011) reported that biotic and water deficit stress in plants promote the biosynthesis of plants' secondary metabolites and bioactive compounds. Therefore, in this study we conducted studies to confirm that suc signaling by the absorption of exogenous suc via roots affected to enhance bioactive compounds, and to determine whether they were induced by subsidiary stress, such as osmotic and biotic stress.
Materials and Methods
1. Plant Materials, Growth Conditions, and Treatments
To identify whether suc treatment promoted water deficit stress and enhanced bioactive compounds, Mugwort (Artemisia princeps Pampanini cv. Sajabal) cuttings were taken that contained a growth point on the stem and rootone (Rootone; ISK, Japan) was applied to the cut node. After that cuttings were placed in 105 cell trays with soil medium (Myung-Moon; Dongbu Hannong, Seoul, Korea) and rooted in a growth chamber under the following environmental conditions: 22°C air temperature, 84% relative humidity, 150 µmol·m-2·s-1 photosynthetic photon flux density (PPFD), and a 12-h light period. After 17 days, 174 rooted mugwort plants were washed with flowing water to remove the soil medium and then transplanted into deep-flow technique (DFT) system (29.5 × 20.2 × 22.5 cm, L × W × H) and cultivated with 4 weeks in a growth chamber (DS-51GLP, Dasol Scientific, Hwaseong, Korea) with the following environmental conditions; 20℃ air temperature, 70 ± 5% relative humidity, 150 ± 5 µmol·m-2·s-1 PPFD, 12-h light period. A Hoagland nutrient solution (pH 5.8, EC 1.1 dS·m-1) was used during cultivation and 10 mM, 30 mM, and 50 mM of suc (D(+)-Sucrose; SAMCHUN, Pyeongtaek, Korea) and man (D-Mannitol; SAMCHUN, Pyeongtaek, Korea) treatments were applied to the nutrient solution for 3 days. Every 2 weeks and before the treatment, the nutrient solution was replaced.
To determine whether biotic stress induced by microorganism proliferation caused by suc treatment affected the bioactive compounds of mugwort. The samples of mugwort were prepared by the same method as described in above experiment and rooted in a growth chamber with the following conditions: 20℃ air temperature, 70 ± 5% relative humidity, 12-h light period, 150 ± 5 µmol·m-2·s-1 PPFD. After 23 days, the rooted mugwort were washed to remove the soil medium (Myung-Moon) and then 101 rooted mugwort plants were transplanted into the DFT system in a growth chamber (20℃ air temperature, 70 ± 5% relative humidity, 150 ± 5 µmol·m-2·s-1 PPFD, 12-h light period) and cultivated for 3 weeks. A Hoagland nutrient solution (pH 5.8, EC 1.1 dS·m-1) with microorganism and/or 30 mM of suc [D(+)-Sucrose; SAMCHUN] for 3 days. The solution of suc 30 mM with the nutrient solution from above experiment (comparison with osmotic stress experiment) was diluted 104 times with sterilized water, and then inoculated into the NA medium (Nutrient Agar; MB cell, Seoul, Korea). Two kinds of microorganism were obtained by incubation (incubator, BF-125MIL, BioFree, Seoul, Korea) for 4 days (Fig. 1). The obtained microorganism was separated from the medium by an inoculation loop, diluted in distilled water, and mixed with Hoagland nutrient solution (pH 5.8, EC 1.1 dS·m-1). The nutrient solution was replaced before the treatment.
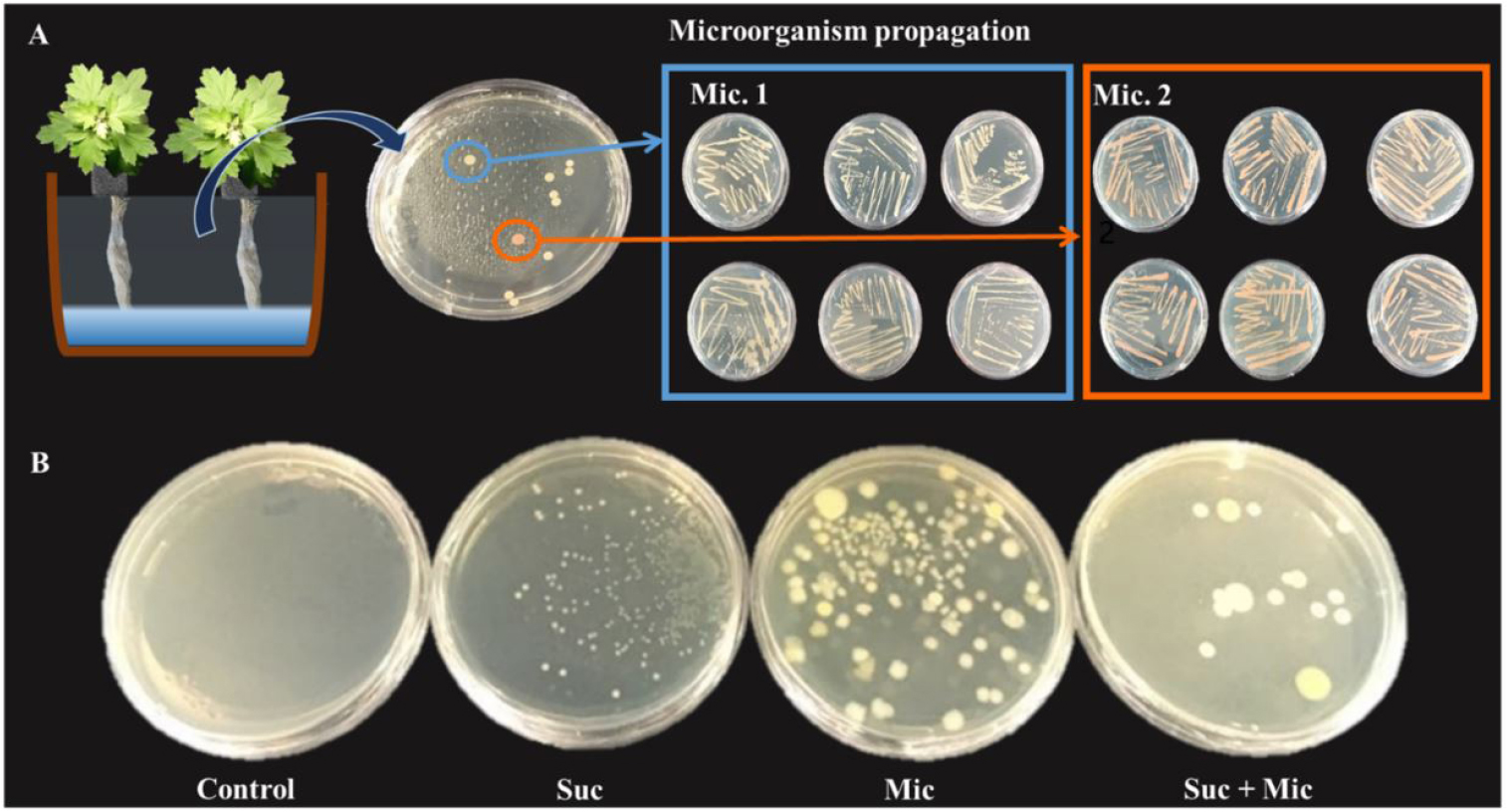
Fig. 1.
Microorganism cultivation from collected nutrient solution treated with sucrose 30 mM for 3 days in the DFT system. Propagation of two types of microorganism 1 (Mic. 1) and microorganism 2 (Mic. 2) used in this study (A) and degree of microorganism cultivated from collected nutrient solution treated with sucrose 30 mM and/or microorganism after 3 days of treatment (B).
2. Shoot Fresh Weight and Leaf Water Potential
Shoot fresh weight of mugwort was measured every day for the 3 days of treatment. An electronic scale (Si-234, Denver Instrument, Bohemia, NY, USA) was used to measure the shoot fresh weight of mugwort. The samples of mugwort were stored in a deep freezer (IU1786D, Thermo Fisher Scientific, Marietta, OH, USA) at -70℃ until analysis. To confirm whether the suc or man induced water deficit stress, leaf water potential was measured using a water potential meter (WP4; Decagon Devices, Pullman, WA, USA) at 3 days of treatment. Leaf discs of 13 mm diameter were obtained from the fourth to fifth node of each plant using a cork-bore and placed in a plastic cup. Before measuring, the cup was equilibrated at 25℃ for 10 min.
3. Sugar Content
To extract sugars, collected mugwort stems and leaves were stored at -70℃ in a deep freezer (IU1786D, Thermo Fisher Scientific, Marietta, OH, USA) until analysis. The samples (0.5 g) were homogenized (PT 2500 E, Kinematica, Luzern, Switzerland) with 3-mL of hot deionized water (70℃). The extracts were incubated for 60 min at 70℃ and centrifuged at 15,000 × g for 10 min at 20°C. The supernatant of the extract was concentrated by a rotary evaporator (N-1000; EYELA, Tokyo, Japan) with a hot water bath (60℃) and 1-mL of deionized water was added before sonication (SK5210HP, Hangzhou Nade Scientific Instrument Co., Ltd., Zhejiang, China). Before analysis, the solution was passed through the 0.45-μm UHP syringe filter (SPU4513-1, Woongki, Seoul, Korea). A high-performance liquid chromatography (YL9100, Young Lin Instrument Co., Ltd, Anyang, Korea) system equipped with a YL9101 vacuum degasser, YL9101 quaternary pump, YL9131 column compartment, and auto sampler were used for sugar analysis (fructose, glucose, and sucrose). For separation of sugars, a NH2P-50 4E column (4.6 × 250 mm, 4.6 × 250 mm, Shodex Asahipak, Showa Denko, Kanagawa, Japan) was used and the temperature of the column was maintained at 35℃. The mobile phase was used with 75% of acetonitrile in water with a flow rate 0.5 mL·min-1. The refractive index detector (Shodex Ri-101; Showa Denko, Kanagawa, Japan) was used and the total run time was 30 min. Standards of fructose [D-(‒)- Fructose; Sigma-Aldrich, St. Lois, Mo, USA], glucose [D-(+)-Glucose; Sigma-Aldrich], and sucrose [D(+)-Sucrose; Sigma-Aldrich] were used.
4. Total Phenolic Content, Total Flavonoid Content, and Antioxidant Capacity
Total phenolic content and antioxidant capacity of mugwort leaves were determined by the method described by Lee et al. (2013), which was modified into the Folin-Ciocalteu colorimetric method (Ainsworth and Gillespie, 2007), and by the 2,2′-azino-bis-3-ethylbenzothiazoline-6-sulfonic acid (ABTS; Sigma-Aldrich) method (Miller and Rice-Evans, 1996), respectively. Fresh mugwort leaves (0.2 g) were powdered by grinding in a mortar with liquid nitrogen. Ground leaves were mixed with 3-mL of 80% acetone and the solution was transferred into 2-mL microtubes. The extracts were incubated in a refrigerator at 4℃ and -20℃ under dark conditions for 12-h to analyze total phenolic content and antioxidant capacity, respectively. The subsequent analysis was performed as described by Son and Oh (2013). Total phenolic content and antioxidant were expressed as gallic acid equivalent (GAE) per gram of fresh weight and trolox (mM) equivalent antioxidant capacity (TEAC) per gram of fresh weight.
Total flavonoid content was determined by the method of Dewanto et al. (2002) with slight modification. A fresh sample (0.2 g) was mixed with 3-mL of 70% ethanol (pH 3.2 by formic acid) and incubated in an ultrasonic sonicator (SK5210, Hanzhou Nade Scientific Instrument Co. Ltd., Zhejiang, China) for 15 min and then stored in a refrigerator 4℃ under dark conditions for 12-h. The extract was centrifuged 3000 × g for 5 min and the supernatant was used for the analysis. The supernatant (250 µL) was mixed with distilled water (1.25-mL) and 5% NaNO2 (75 µL) and reacted for 5 min. After that, 10% AlCl3 (150 µL) was added to the mixtures. After 6 min, 1M NaOH (500 µL) and distilled water (275 µL) were added to reacted mixtures. After 5 min, the optical density of the mixtures was measured by a spectrophotometer (UV-1800, Shimadzu, Kyoto, Japan) at 510 nm. Total flavonoid content was expressed as (+)-catechin (mg) ((+)-catechin hydrate, Sigma-Aldrich) equivalent per gram of fresh weight.
5. Protective effect of cytotoxicity in mugwort
To analyze cytoprotective effects of suc-treated mugwort extracts, fresh mugwort samples were extracted with 100-mL of 100% methanol in a shaker (Eyela Model MMS-300; Tokyo Rikakikai, Tokyo, Japan) at room temperature for 24-h and the extract was filtered through Toyo No. 2 filter paper (Toyo Ltd., Tokyo, Japan). To remove methanol, the filtrate was evaporated at 35°C. The dried extracts were dissolved in dimethyl sulfoxide (DMSO; Sigma-Aldrich) at 50 mg·mL-1 and stored at -20°C until analysis.
HepG2 cells (Korean Collection for Type Cultures; Daejeon, Korea) were cultured in Dulbecco's Modification of Eagle's Medium (DMEM) supplied with 10% heat-inactivated FBS (fetal bovine serum; Gibco BRI, Gaithersburg, MD, USA), 100 U·mL-1 penicillin, 100 µg·mL-1 streptomycin, and 5.5 mM D-glucose (Sigma-Aldrich) in a humidified incubator with 5% CO2 at 37°C.
Cell viability was measured using the MTT assay (Mosmann, 1983). HepG2 cells were placed in 96-well plates at a density of 1 × 105 cells per well and incubated for 24-h. Next, the cells were treated with 100 µg·mL-1 of the sample of mugwort extract. For ethanol-induced cytotoxicity, the cells were treated with EtOH (3%) after 24-h. For tert-butyl hydroperoxide (t-BHP)-induced oxidative stress in HepG2 cell, the culture medium containing the samples was discarded after 12-h and the cells were treated with t-BHP (500 µM). After 24-h, 0.5 mg·mL-1 MTT reagent (Sigma-Aldrich), dissolved in FBS-free DMEM, was added to each well. After 2- and 4-h of incubation for ethanol and t-BHP-induced cytotoxicity, respectively, the culture medium was removed, and the intracellular formazan product was dissolved in DMSO (Sigma-Aldrich). The optical density was measured at 550 nm using a spectrophotometer (Beckman Instruments Inc., Fullerton, CA, USA).
6. Data Analysis
Three identical DFT hydroponic systems with six plants were used for each treatment in a completely randomized design. Five replicates per treatment were used for shoot fresh weight, total phenolic content, antioxidant capacity, and total flavonoid content. Four replicates were performed for each treatment for leaf water potential and sugar analysis. Cell cytotoxicity tests were conducted in triplicate. Analysis of variance was performed using the Statistical Analysis System (SAS 9.2; SAS Institute, Cary, NC, USA) and Duncan’s multiple range test was used to compare means. Differences were considered significant at p < 0.05.
Results and Discussion
Comparison with Osmotic Stress
1. Shoot Fresh Weight and Leaf Water Potential
To determine whether the water deficit stress was caused by suc treatment, the shoot fresh weight and leaf water potential were measured. Shoot fresh weight of man and suc treatments were not significantly different than that of the control for 3 days of treatment (Table 1). In our previous study, 3 days of suc treatment was a temporary treatment, which was not sufficient to increase mugwort growth.
Table 1.
Shoot fresh weight of mugwort plants treated with mannitol and sucrose for 3 days at 4 weeks of transplanting.
Treatment | Shoot fresh weight (g) | ||
1 day | 2 days | 3 days | |
Controlz | 2.8 ± 0.5y | 2.5 ± 0.2 | 2.7 ± 0.3 |
Mannitol 10 mM | 2.8 ± 0.8 | 1.5 ± 0.5 | 1.8 ± 0.7 |
30 mM | 2.4 ± 0.5 | 4.6 ± 0.6 | 3.2 ± 0.8 |
50 mM | 3.4 ± 0.7 | 3.4 ± 1.0 | 4.3 ± 1.2 |
Sucrose 10 mM | 3.7 ± 0.4 | 3.7 ± 0.8 | 3.7 ± 0.4 |
30 mM | 2.4 ± 0.6 | 3.0 ± 0.5 | 3.3 ± 0.2 |
50 mM | 4.0 ± 0.2 | 3.9 ± 0.7 | 2.7 ± 0.5 |
Significancex | NS | NS | NS |
Man in the medium increased osmotic pressure and induced water deficiency in the plants (Tholakalabavi et al., 1994; Ikeda-Iwai et al., 2003; Slama et al., 2007). Leaf water potential was not significantly different among the control, man, and suc treatment (Fig. 2). However, leaf water potential of man 50 mM was -14.4 MPa, which was 1.2 times lower than the man 10 mM. Man treatment showed a tendency depending on the concentration in the leaf water potential. On the other hand, the suc 10 mM and suc 50 mM treatments did not exhibit patterns and it was difficult to conclude that suc induced the water deficit stress by osmosis.
2. Total Phenolic Content, Total Flavonoid Content, and Antioxidant Capacity
In this study, we identified the effect of osmotic stress and suc signaling on the increase in bioactive compounds. Both man and suc treatments were effective in the enhancement of bioactive compounds of mugwort after 4 weeks of transplanting. For 3 days of man and suc treatment, the total phenolic content, total flavonoid content, and antioxidant activity of the mugwort increased, but they exhibited different patterns (Fig. 3). At 3 days of treatment, total phenolic content of man 50 mM was significantly 1.4-fold higher than that of the control, whereas the total phenolic content of suc 30 mM was 1.4-fold higher than that of the control, although there was no significant difference at 1 day of treatment (Fig. 3A and 3B).
In case of total flavonoid content, the increased pattern was quite different between man and suc treatments. This implies that the activation mechanism of each treatment was different. At 1 day of treatment, man 10 mM and 30 mM were significantly 1.8-fold and 1.9-fold higher than the control, respectively and man 50 mM was 1.8-fold higher compared to the control at 2 days of treatment (Fig. 3C). Regarding suc treatment, suc 10 mM and 30 mM had 2-fold and 2.3-fold higher total flavonoid content than that of the control only at 3 days of treatment (Fig. 3D). Antioxidant capacity of man 50 mM was increased 1.4-fold higher than that of the control at 2 days of treatment (Fig. 3E). Antioxidant capacity of suc 30 mM was significantly higher (1.5-fold) than that of the control at 1 day of treatment; however, at 2 and 3 days of treatment were not significantly different (Fig. 3F).
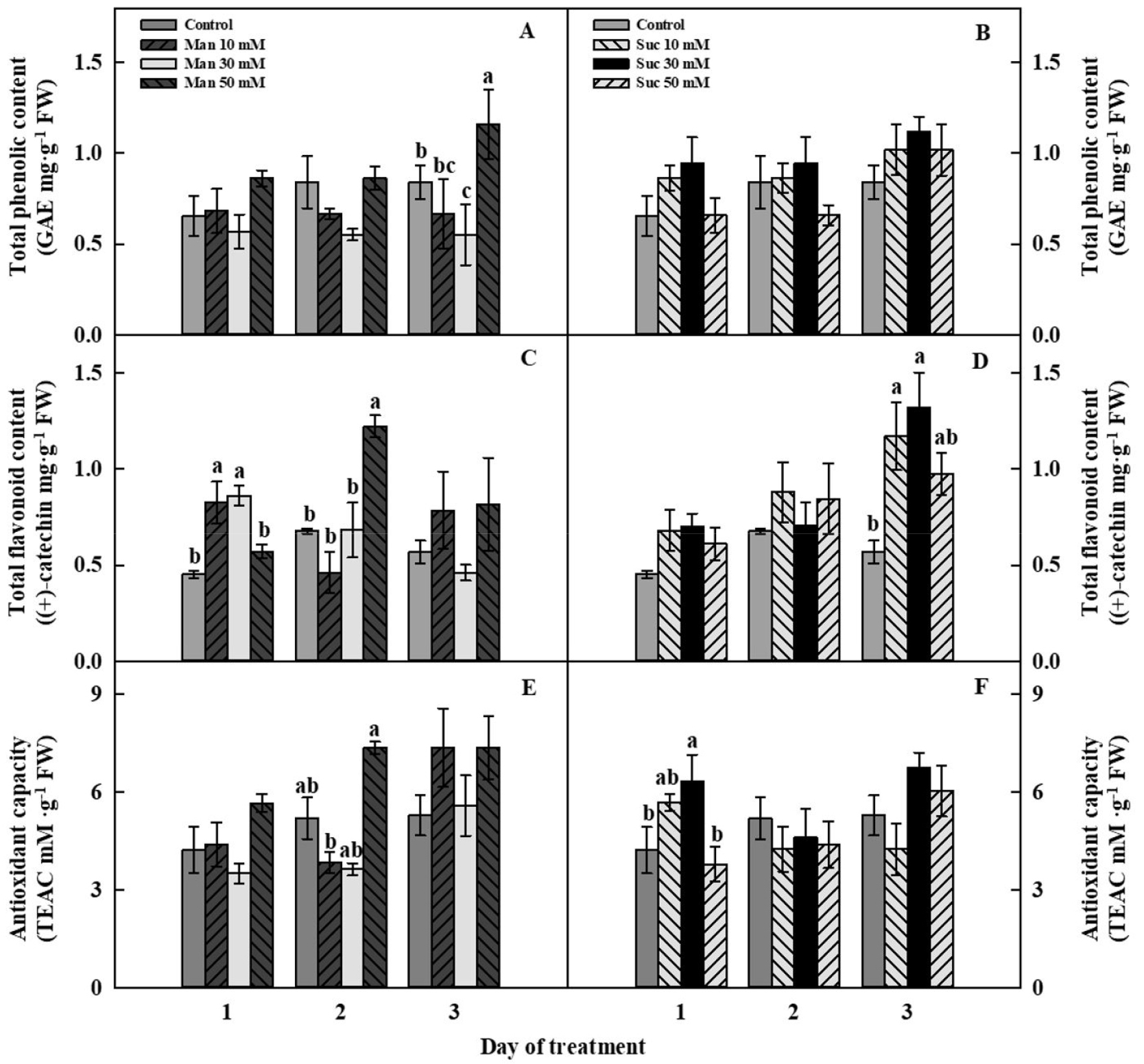
Fig. 3.
Total phenolic content (A, B), total flavonoid content (C, D), and antioxidant capacity (E, F) of mannitol- (A, C, E) and sucrose- (B, D, F) treated mugwort plants for 3 days of treatment after 4 weeks of transplanting. The data indicate means ± SE (n = 5). Different letters above bars indicate significant difference at p < 0.05.
Man treatments improved the content of bioactive compounds of mugwort because of a mild water deficiency. Typically, plant cells produce 10-fold higher ABA, a plant hormone in response to a water deficit stress compared to normal plant cells (Amrhein et al., 2013). The increased level of ABA in plants inhibits the photosynthetic rate and shoot growth and also produces reactive oxygen species (ROS) that induce toxicity and damage of plants cells (Lee et al., 2022). However, most plants have detoxification mechanisms for ROS through the production of enzymatic and non-enzymatic antioxidants. Non-enzymatic antioxidants are known as bioactive compounds that can promote human health (Briskin, 2000; Lee et al., 2022). In previous studies, mild water deficit stress increased the content of chicoric acid of lettuce, anthocyanins of dropwort, and ascorbic acid in the leaves of wheat (Nayyar and Gupta, 2006; Oh et al., 2010, Lee et al., 2022).
Guo et al. (2011) watered broccoli sprouts with 176 mM of suc and man in a petri dish for 5 days. The results showed that both man and suc treatments increased the contents of total phenolics and glucosinolates. However, the activity of myrosinase (catalyzes the hydrolysis of glucosinolates) was significantly decreased and the activity of PAL enzyme was significantly increased only in suc treatment. These results indicated that, unlike man, suc acts as a signaling molecule to induce the biosynthesis of bioactive compounds in plants.
Since suc is an essential component of plants, suc concentrations above specific threshold are required for suc signaling to appear (Pollock et al., 2003). Excess sugar in plants can occur not only by exogenous suc supply, as in our experiment, but also by the stress response. For example, water deficit stress is a strategy to prevent water loss by inducing osmotic adjustment by increasing compatible solutes, such as suc, glu, fru, inositol, and proline. Kerepesi and Galiba (2000) reported that water-soluble carbohydrates, such as suc, glu, and fru could be used as markers to identify resistant varieties of wheat. In addition, the activity of sucrose phosphate synthase, which is a primary sucrose biosynthetic enzyme, was induced by low temperature treatment (5℃) of spinach, and thereby, suc, glu, and fru contents increased from 10 to 20-fold that of the control (25℃) (Guy et al., 1992). Tognetti et al. (2013) reported that the supply of exogenous suc of the plant mimics the effect of low temperature and high-light intensity, which increases the anthocyanin content and antioxidant activity to eliminate ROS in plants. Regarding excess sugars in plants, the increase in sugar content by exogenous supply of suc and the stress response are similar with each other. Therefore, an increase in bioactive compounds induced from plant stress can be caused by the supply of exogenous suc.
3. Protective Effect of Cytotoxicity
Treatment of ethanol and t-BHP, which induce damage by alcohol and oxidative stress, to HepG2 cells (liver cells) resulted in the toxicity of hepatocytes. Mugwort is a medicinal plant containing various flavonoids, such as eupatilin, jaceosidin, eupafolin, apigenin, and hispidulin (Choi et al., 2007; Chung et al., 2010). In particular, eupafolin and hispidulin are known to be effective in the protection of liver cells because of antioxidant activity (Chung et al., 2010; Gao, 2014; Jabeen et al., 2016). In the present study, liver cell viability was reduced by 52% and 46%, respectively, by ethanol and t-BHP-induced liver cell damage (Fig. 4A and 4B). However, the pretreatment of suc 50 mM treated mugwort extract resulted in approximately 1.7-fold higher cell viability than the ethanol-treated liver cells at 1 day to 3 days of treatment (Fig. 4A). Regarding t-BHP-induced liver cell damage, suc 50 mM was resulted in 1.6-fold higher cell viability than that of t-BHP-treated liver cells at 1 day to 3 days of treatment (Fig. 4B).
Yang et al. (2012) reported that the enhancement of caffeic acid, one of the liver protecting bioactive compounds in perilla leaves, was confirmed in the suc-treated perilla leaf extract. Suc (6%)-treated perilla leaf extract had high caffeic acid and thereby induced a protective effect against liver cell damage by t-BHP. In the present study, the mugwort extract treated with suc 50 mM was more effective in the protection against hepatocyte damage from ethanol and t-BHP than suc 30 mM and had higher phenolic antioxidants. This is consistent with our previous results. This indicates that suc 50 mM-treated mugwort may generate suc signals for the biosynthesis of bioactive compounds that can protect damaged liver cells.
Comparison with Biotic Stress
1. Shoot Fresh Weight and Sugar Analysis
For 3 days of suc 30 mM and/or microorganism treatments in the DFT system, there was no significant difference (Table 2). However, at 3 days of treatment, suc 30 mM and microorganism with suc 30 mM (Suc + Mic) treated mugwort were significantly increased sugar content by 1.8-fold and 1.6-fold higher than that of the control, respectively, and 2.2-fold and 1.9-fold higher compared to the microorganism treatment, respectively (Fig. 5). These results indicate that the nutrient supply of suc was absorbed through the roots and induced sugar accumulation in the mugwort. Suc is absorbed into the plants by the sucrose transporter (SUT) via roots (Veillet et al., 2016). It is transported through the phloem, and then enters the cytoplasm to generate the signaling (Ruan, 2014). Shin et al. (2013) confirmed that sucrose transporter 1 (SUT1) of Arabidopsis root was activated by suc (2.16%) in MS medium. The results of the present study also showed that suc may be absorbed by the sucrose transporter (SUT) of the mugwort roots resulting in increased sugar content of mugwort plants.
Table 2.
Shoot fresh weight of mugwort plants treated with sucrose 30 mM and/or microorganism for 3 days at 3 weeks of transplanting.
Treatment | Shoot fresh weight (g) | ||
1 day | 2 days | 3 days | |
Controlz | 2.7 ± 0.6y | 3.9 ± 0.6 | 4.2 ± 0.8 |
Sucrose 30 mM | 3.3 ± 1.5 | 3.6 ± 0.7 | 2.8 ± 0.6 |
microorganism | 2.7 ± 0.7 | 2.3 ± 0.6 | 3.0 ± 0.6 |
Sucrose 30 mM with microorganism | 2.3 ± 0.8 | 2.6 ± 0.5 | 2.8 ± 07 |
Significance | NS | NS | NS |
2. Total Phenolic Content and Antioxidant Capacity
Microorganism can enter through the water, humans, or plant materials to the controlled environment of cultivation systems (Gilberto, 2011). As a carbon source, suc treatment can accelerate growth of microorganism, such as fungi and bacteria. Therefore, suc treatment in the DFT system can induce biotic stress in plants. Biotic stress can stimulate the biosynthetic pathway of secondary metabolites in plants. Bednarek et al. (2009) reported that the accumulation of glucosinolates was confirmed as an antifungal defense response when Arabidopsis was infected by pathogens. In addition, Zubek et al. (2012) reported that the contents of hypericin and pseudohypericin, which are pharmacological components of St. John’s-wort, were enhanced by arbuscular mycorrhizal (AM) fungi.
In this study, microorganism was obtained through the cultured medium gained from the solution of suc 30 mM treated nutrient solution for 3 days after 4 weeks of transplanting, and then microorganism treated with suc 30 mM were individually treated to the nutrient solution. Total phenolic content of suc 30 mM and microorganism with suc 30 mM (Suc + Mic) treatments were 1.2-fold and 1.3-fold higher than that of the control at 3 days of treatment (Fig. 6A). Antioxidant capacity at 3 days of treatment were non-significantly different; however, suc 30 mM was 1.3-fold and 1.1-fold higher than that of the control and microorganism treatment, respectively. The microorganism with suc 30 mM treatment had 1.5-fold and 1.3-fold higher antioxidant capacity than that of the control and microorganism treatment, respectively (Fig. 6B). These results indicate that the suc signaling effect might have stimulated the enhancement of bioactive compounds in mugwort; however, biotic stress caused by microorganism or the interaction of AM with mugwort did not stimulate these changes.
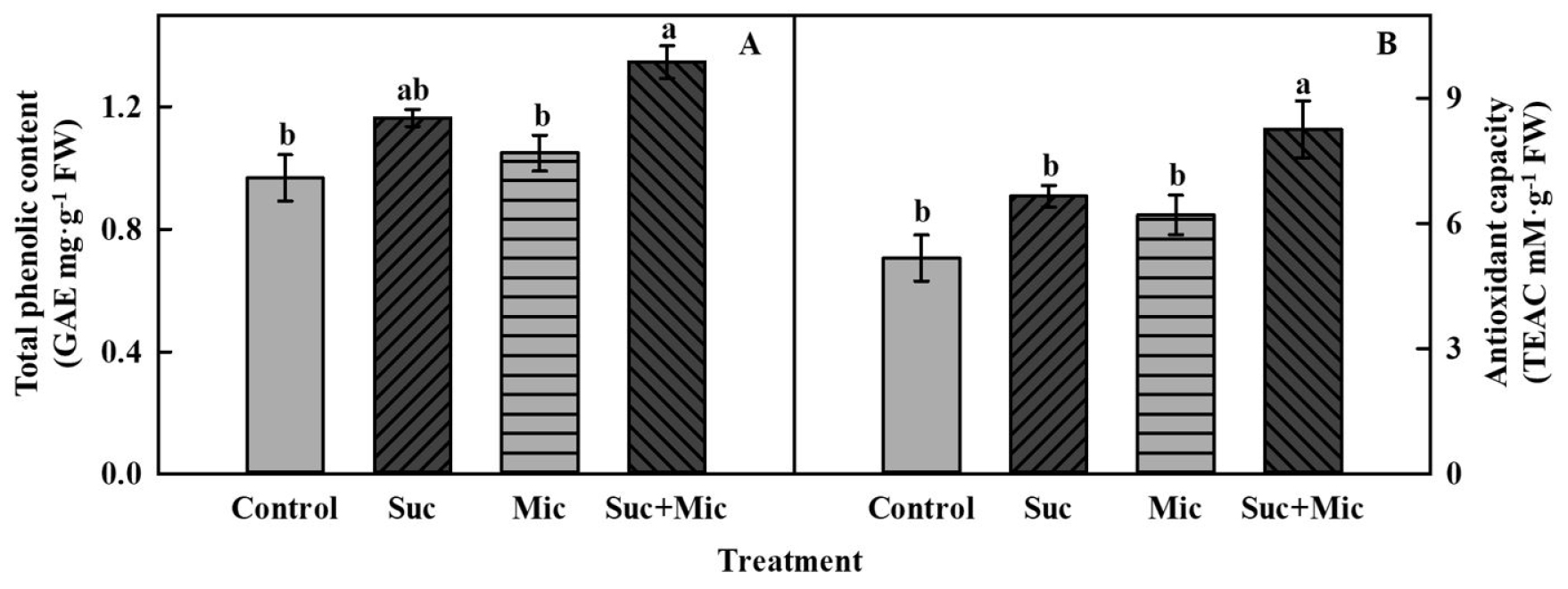
Fig. 6.
Total phenolic content (A) and antioxidant capacity (B) of mugwort plants treated with sucrose 30 mM (Suc) and/or microorganism (Mic) at 3 days of treatment after 3 weeks of transplanting. The data indicate means ± SE (n = 5). Different letters above bars indicate significant difference at p < 0.05.
Conclusion
Our results suggested that the enhancement of bioactive compound by suc treatment may result from different pathways from that induced by osmotic stress. In addition, in the hydroponic system, suc treatment induced growth of microorganism, but did not affect the production of bioactive compounds in mugwort. Thus, we conclude that suc signaling was induced by absorption of suc via roots leading to synthesis of bioactive compounds and thereby represented the protective effect of suc-treated mugwort on liver cells damaged by ethanol and t-BHP.